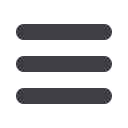
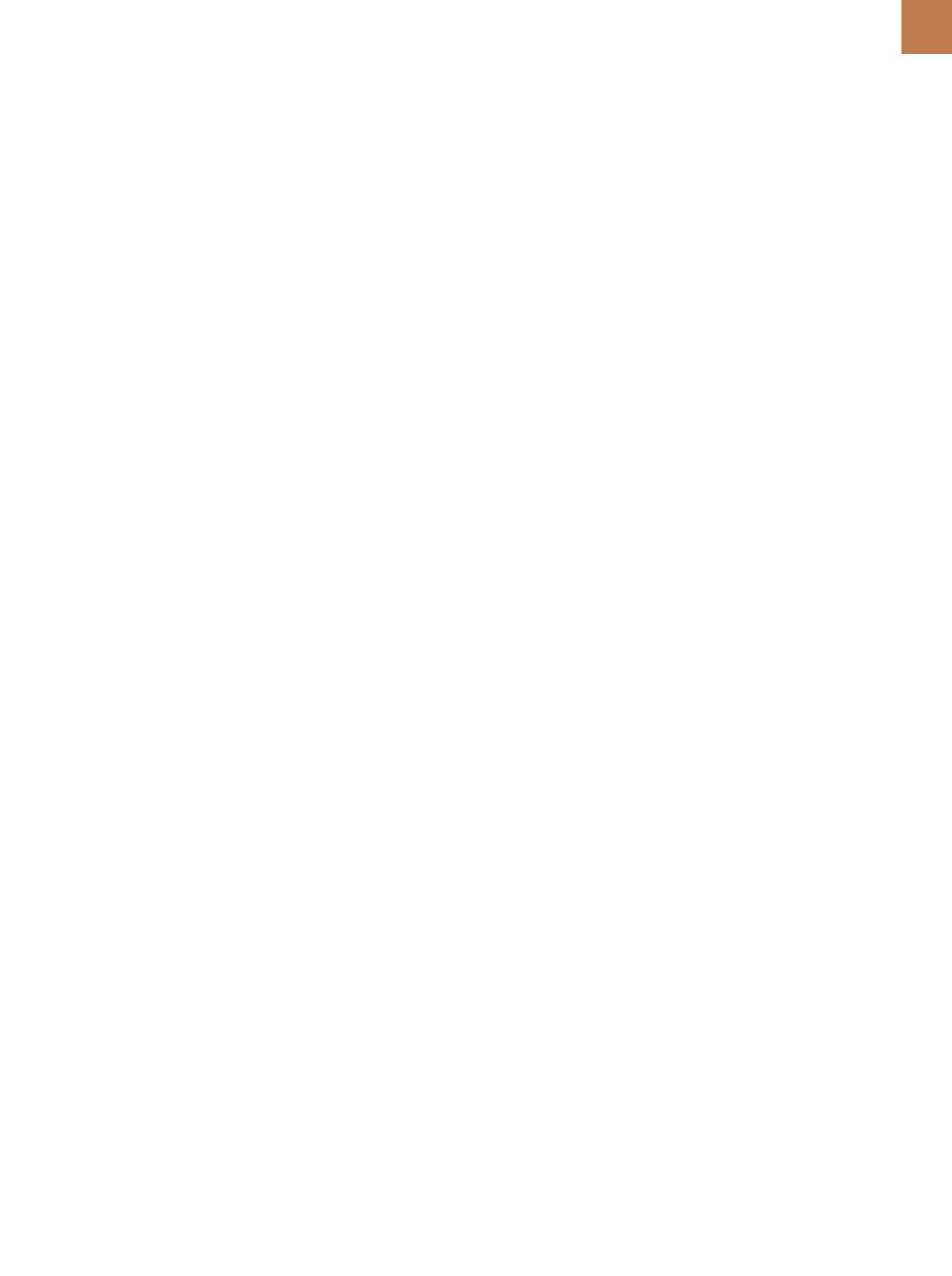
A D V A N C E D
M A T E R I A L S
&
P R O C E S S E S |
N O V E M B E R / D E C E M B E R
2 0 1 6
1 7
A
nuclear reactor core is an incredi-
bly demanding environment that
presents several unique chal-
lenges for materials performance. Mate-
rials in modern light water reactor (LWR)
cores must survive several decades in
high-temperature (300-350°C) aqueous
corrosion conditions while being subject
to large amounts of high-energy neu-
tron irradiation. Next-generation reactor
designs seek to use more corrosive cool-
ants (e.g., molten salts) and even greater
temperatures and neutron doses. The
high amounts of disorder and unique
crystallographic defects and micro-
chemical segregation effects induced by
radiation inevitably lead to property deg-
radation of materials. Maintaining struc-
tural integrity and safety margins over
the course of the reactor’s service life thus
necessitates theability tounderstandand
predict these degradation phenomena in
order to develop new, radiation-tolerant
materials that can maintain the required
performance in theseextremeconditions.
Historically, performing the neu-
tron radiation damage characterization
necessary to assess radiation tolerance
and performance has faced two primary
challenges. First, preventing undue
radiation exposure and contamination
is problematic, as neutron absorption
tends to generate radioactive isotopes
in a material, which can make materi-
als handling hazardous without spe-
cialized equipment and facilities. As
such, the required sample preparation
for post-irradiation examination (PIE)
often needs to be performed in shielded
hot cells and is limited to functions that
can be performed therein. Practically
speaking, this often precludes access
to advanced microstructural character-
ization tools such as transmission elec-
tron microscopes (TEMs) and makes
even basic characterization procedures
much more costly. Second, neutron
irradiation experiments typically have
a long turnaround time, as achieving
the doses necessary to produce end-
of-life microstructure and mechanical
properties in a material requires that
specimens be exposed in a commercial
reactor core for a length of time equiva-
lent to the expected service lifetime. As
a result, researchers in this field often
use high-energy ion beam accelerators
in attempts to emulate neutron dam-
age processes at a much faster rate
without inducing radioactivity in speci-
mens. Although these methods provide
valuable insight into a material’s irradi-
ation response, they are not completely
predictive due to differences in the
mechanism in which charged particles
and neutrons impart energy to the crys-
tal structure
[1]
.
Fortunately, modern PIE exper-
iments can make use of specialized
high-flux test reactors and dedicated
characterization facilities in order to
circumvent some of these issues. The
Advanced Test Reactor (ATR) at Idaho
National Laboratory (INL) and the High
Flux Isotope Reactor (HFIR) at Oak
Ridge National Laboratory (ORNL) are
examples of two specialized nuclear
reactor designs in the U.S. capable of
inducing neutron damage structures
at much greater rates compared to
commercial LWRs. Further, access to
dedicated, radiation-friendly character-
ization laboratories available through
user facilities such as the Nuclear Sci-
ence User Facilities (NSUF, atrnsuf.inl.
gov) can provide the resources and per-
sonnel necessary to limit occupational
exposure and prevent the spread of
radioactive contamination.
An important tool within these
user facilities and radiological charac-
terization laboratories is the focused
ion beam (FIB), used to prepare spec-
imens for both TEM and atom probe
tomography (APT) characterization.
Sample preparation through FIB tech-
niques has been particularly impactful
in working with radioactive materials
due to the significantly smaller working
sample volumes leading to increased
scientific yield gained from individual
experiments. Using carefully performed
FIB lift-out techniques, a highly radio-
active bulk specimen can be reduced
to such a minute volume that it no lon-
ger poses a significant exposure risk.
As even a single bulk sample can have
significant associated cost after irradia-
tion in a reactor like HFIR, extracting the
maximum amount of information from
these samples is crucially important. As
FIB can produce targeted, site-specific
analysis and allows for multiple TEM
and APT specimens to be prepared from
a single bulk sample, it is extremely
useful for maximizing the scientific and
monetary value of a single bulk irradi-
ated sample.
Some examples of radiation-
induced microstructural defects being
studied using these techniques include
dislocation loops, cavities, gas bubbles,
segregation, and precipitates. Charac-
terization of these nanoscale features
necessitates advanced microscopy
equipment and techniques that often
require special considerations when
performing data collection or analysis
for radioactive materials. This article
illustrates the use of these advanced
techniques to characterize precipitates
in neutron-irradiated Fe-Cr-Al alloys at
ORNL, which have extensively utilized
the aforementioned research reactors
and user facility characterization lab-
oratories. However, the techniques
described here can be readily extended
to other materials as well.
CHARACTERIZATION OF
IRRADIATED Fe-Cr-Al
Fe-Cr-Al alloys are being consid-
ered as a possible replacement for
Zr-based fuel cladding materials cur-
rently used in commercial LWRs for
increasing safety margins and enhanc-
ing accident tolerance as part of the
DOE’s Advanced Fuels Campaign
[2]
.
Similar to other high-Cr ferritic alloys,
Fe-Cr-Al alloys have excellent high tem-
perature aqueous corrosion and radia-
tion-induced swelling resistance
[3]
, but
are susceptible to radiation-induced
hardening and embrittlement due to
the precipitation of nanoscale Cr-rich
α
ʹ phase precipitate particles at tem-
peratures below 475
°
C. Though the
kinetics for formation of this phase at
LWR-relevant temperatures are slow,
this process has been shown to be
accelerated by neutron-induced radi-
ation damage
[4]
. An understanding
of how the formation of this phase is
affected by composition and how the
precipitate microstructure evolves with
radiation dose is essential in order to
mitigate the embrittlement response in